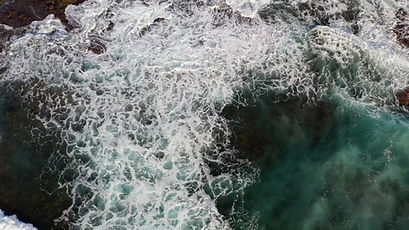
What are we working on?
Data-augmented turbulence modeling
We are working towards the goal of developing generalizable data-augmented turbulence models. Critical components of this approach include: (1) imposition of strictly necessary physical constraints; (2) maintaining consistency between the learning and prediction environments; (3) tightly-coupled inference and learning by constraining the augmentation to be learnable throughout the inference process; (4) meticulous design of a parsimonious set of physics-informed, locally non-dimensional, and bounded features while promoting construction of a one-to-one features-to-augmentation map and (5) localized learning to maintain explicit control over feature space to sensitize the augmentation function behavior only in the vicinity of available data. The vision is to develop a complete framework for turbulence modeling.
Biofouling
Biofouling on marine vessels is inevitable and typically results in increased drag and performance deterioration. Calcareous hard biofoulings such as mussels, barnacles, and compliant soft biofoulings such as algae cause significant increase in drag of ships, underwater vehicles (UUV’s), and other platforms of naval interest. The complex physical processes that influence biofilm growth depend on several factors such as substrate material, growth duration, fouling species, and ambient conditions. Biofilm growth determines morphological properties of the coated fouling such hardness/stiffness, coverage, film thickness, and filament thickness/length.
Fouled surfaces interact with and alter their external turbulent boundary layer, which influences resistance, sound, controllability and sensor operation. Thus, the hydrodynamics of biofoulings is a complex multidisciplinary problem involving biology, oceanography, material science, structural mechanics and fluid mechanics. The reliable prediction and design of vessels operating in waters of the future necessitates the development of a first-principles approach to understanding and modeling the influence of biofouled surfaces.
Wave energy
Covering 72% of the earth’s surface, oceans and lakes contain vast but untapped renewable marine energy and hold great potential for sustainable energy supply. This includes the ocean waves, tidal and ocean currents, and offshore wind. The widespread adoption of marine energy is still in infancy because of convergence challenges in engineering, economic, and socio-environmental perspectives. A wave energy converter (WEC) usually includes four key components: wave capture structure to convert the hydrokinetic energy into mechanical energy, power takeoff (PTO) to convert mechanical energy into electricity, power electronics for grid or micro-grid connection, and control system for optimal operation. We conduct research into both the individual components as well as system-level co-design paradigms.
Machine learning & data assimilation
Data assimilation aims to bridge models and reality. It originates from weather forecasting, where real-world data was used to reduce the uncertainty in model parameters and thereby improve weather predictions. In Fluid Dynamics, data assimilation offers the promise to enrich coarse experimental data with simulation results to reconstruct the entire three-dimensional, time-dependent flow fields and applications such as guiding strategic sensor placements in experimental design. We conduct research into data assimilation to develop experimental measurement techniques such as particle-tracking velocimetry (PTV), to predict quantities that are very difficult to measure such as pressure and to obtain near-wall measurements from outer layer data. Fundamental advances in the methodology ranging from adjoint-based approaches to machine-learning approaches are under development.
Materials
Next-generation materials could revolutionize the study and control of fluid-surface interaction, hydroacoustics, and other naval applications. For example, simple cut and fold patterns can transform 2D sheets into 3D shapes with complex behaviors. We conduct research into materials, metamaterials, and compliant mechanisms generated in this manner for achieving certain mechanical properties. Integration with active electronic and optoelectronic materials and devices can lead to superior functional and techno-economic performance in energy conversion, storage, and wearable sensors.
We conduct research into achieving cost-effective construction for the next generation of naval structures. A central theme is the implementation of smart modular design for neat assembly from piece-part level all the way to final ship units or blocks. We also work on the associated underpinning mechanics principles including novel dissimilar materials direct joining and un-joining techniques for achieving both optimal structural lightweighting and multi-functionality.
Physics-based multi-scale modeling of coupled reaction mechanisms that span atomistic to cell and system design levels can accelerate the incorporation of new materials and new materials approaches into energy storage devices and systems for naval applications. We are developing Integrated Computational Materials Engineering (ICME) methods that can be efficiently verified against experimental measurements, and used to guide novel material and microstructure modeling and discovery. The key principle is the development of efficient and flexible dynamical models which are constrained by known equilibrium thermodynamic behavior.
Complex hydrodynamics & flow control
Cavitation, the formation of vapor cavities in liquid flows and the subsequent oscillation of these bubbles, has long been known to lead to negative outcomes in hydrodynamic flows, including noise, vibrations, performance losses, and even structural damage. Cavitation also has medical applications such as lithotripsy and blast-induced traumatic brain injury. Cavitation originates from sub-micron nuclei and, if severe enough, proceeds the span the entire chord of propeller blades. We conduct research into the development of high-fidelity approaches to compute and measure turbulent cavitating flows, including their interaction with material surfaces.
Turbulence features prominently in naval and marine flows. Our research focuses on fundamental advances in direct numerical simulation (DNS) and large-eddy simulation (LES) and understanding of flow physics that allow the reliable simulation of multi-physics turbulent flows. Key pacing items in this endeavor are numerical algorithms, sub-grid models, physical models, high performance computing and big data analysis. Applications include flow over complex geometries, superhydrophobic surfaces and fluid-structure interaction.
Many aquatic and avian species possess the unique ability to morph their fins or wings, optimizing hydrodynamic efficiency and maneuverability. This intricate fluid-structure interaction offers exciting potential for innovations in flow control. We are exploring new avenues for adaptive flow control in various engineering applications such as smart morphable strategies to dynamically adjust surface topography to influence drag and lift.
Electromagnetics
Estimating the radar characteristics of large objects has diverse applications in industry and remote sensing. For instance, in remote sensing applications, like measuring ocean surface wind speeds and directions, or sea ice thickness, the radar footprint often spans areas much larger than the wavelength. For large objects, the radar response varies based on factors like frequency, radar aspect angle, and range, especially outside the far-field range. Small changes in frequency or incidence angle can cause rapid and unpredictable variations, complicating accurate estimation. We conduct research into statistical models to compute radar responses in dynamic environments, such as traffic scenes, and in complex propagation scenarios, like wave transmission through turbulent media (e.g., rain).